Introduction to Physical Geology |
|
Chapter 3 - Basic Geologic Principles |
3.1
Geology is founded on basic principles that are useful for making many useful observations about the world around us. This chapter presents a mix of information that is essential (fundamental) to all following chapters. This chapter expands on the concepts focused on the solid earth (geology). We begin with a discussion on geologic time and the rock cycle — a graphic and conceptual model to illustrate common rocks and earth materials and the processes that form or change them, over time.
A Focus On Interpretation
Several basic geologic principles can be applied to resolving the order of events leading to the formation of rocks and landscape features. This section presents many basic concepts that are universal to all physical sciences. Geologists use geochronology — the study of the age of rocks, using both absolute dating methods and relative dating methods.
The second half of this chapter focuses on maps and the display of geographic and geologic information in two-dimensional and three-dimensional formats. Geologic maps are essential components to resolving the geologic story (and history) of a region, and serve many useful purposes for society including uses in urban planning, agriculture, health and public safety, mineral resource management, water resources, and much more.
|
Click on thumbnail images for a larger view. |
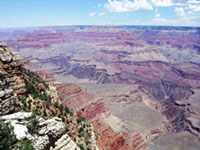
Fig. 3-1. The Grand Canyon in Arizona is perhaps one of the best places to see layers of rock of many ages exposed that reveal information about the regions geologic history. |
3.2
Basic Geologic Principles For Interpreting Landscape Forming Processes
The surface of the Earth is shaped by processes moving materials underground and by processes occurring on the surface under the influence of atmospheric, hydrologic, and oceanographic processes. The logical place to start discussion is to examine the material below our feet. Perhaps most fundamental to understanding the geologic origin of landscapes and earth materials is the vastness of geologic time. It took centuries for the scientific community to compile information about the age of different regions in the planet through the study of rocks, the fossils they contain, and the chronology of events in Earth's history (such as the formation of mountain ranges, changes in coastlines, and the evolution of life). One of the products of this collective investigation over time is the geologic time scale (Figure 3-2). The time scale links measures of time (on the scale of periods ranging from thousands, millions, to billions of years) to established names for those time periods used in publications (reports and maps). The geologic time scale has been continuous refined as new data is collected. (See section 1.10 in Chapter 1 for a discussion on geologic time.)
What Is Bedrock?
Bedrock is the solid rock that occurs beneath soil or alluvium (unconsolidated sediments) that coved the surface of the land in most locations. In some places the bedrock is exposed as rocky outcrops scattered across the landscape, particularly in mountainous areas or along stream canyons. The term outcrop is used to describe a location where bedrock is exposed (or crops out).
Why do landscapes in different areas have unique characteristics? Its all about the bedrock and its history!
Landscapes change from one region to the next because of the composition and character of bedrock changes from place to place.
Geologists attempt to unravel geologic history by studying the geometry of outcrops in an area. Subtle characteristics of undisturbed landscapes often reveal the location of faults, ore deposits, sources of groundwater, and many other features of geologic interest.
|
Fig. 3-2. Geologic Time Scale |
3.3
The Rock Cycle and Rock Classification (revisited)
Charles Lyell (1797-1875) compiled the first geology textbook entitled "Principles of Geology" in which he promoted concepts of the "rock cycle" (Figure 3-3). The rock cycle illustrates the series of events in which a rock of one type is converted to one or more other types, and then possibly back to the original type. The rock cycle is a graphic and conceptual model to illustrate common kinds of rocks and earth materials and the processes that form or change them over time. Figure 3-4 also illustrates the rock cycle—but includes more detail about both rocks and earth materials, and selected geologic features associated with geologic processes. Be sure to examine the arrows on the diagrams! Pathways to rock origins may go several routes—rocks of any kind can be changed in different ways into a variety of other rocks types. It is important to note that tectonic forces within the Earth are responsible for many of the changes to rocks and surface features on the landscape. Tectonic forces are responsible for the gradual uplift of mountain ranges, both on land and on the seafloor, as well as creating basins that flood with lakes or seawater, and fill in with sediments (Figure 3-5).
There are 4 classes of rocks and earth materials: igneous rocks, sediments (which are not rocks), sedimentary rocks, and metamorphic rocks.
Igneous rocks are rocks formed from the cooling and crystallization of molten materials (Figure 3-6). The word igneous applies to any rock or mineral that solidified from molten or partly molten material (referring to magma underground or lava on the surface). The word igneous also applies to the processes related to the formation of such rocks. Igneous rocks includes intrusive rocks (rocks that cooled below the surface) and volcanic rocks formed on the Earth's surface by volcanism. Igneous rocks also form from melting associated with extraterrestrial impacts. Examples of igneous rocks include granite, gabbro, and basalt. Rocks of igneous origin are discussed in Chapter 7.
Sediments are solid fragments of inorganic or organic material that come from the weathering of rock and soil erosion, and are carried and deposited by wind, water, or ice (Figure 3-7). Sediments may be made up of rock fragments ranging is size from boulders down to microscopic particles, and may contain organic matter. Examples of sediment include gravel, sand, silt, clay, mud (mix of sand, silt, and clay), soil, lime mud, and ooze. Sediments are not rocks, but they may become rocks through heating, compaction, and cementation. Sediments are discussed in Chapter 8.
Sedimentary rocks are rocks that have formed over time through the deposition and solidification of sediment, especially sediment transported by water (rivers, lakes, and oceans), ice ( glaciers), and wind (Figure 3-8). Sedimentary rocks are often deposited in layers, and frequently contain fossils. Sedimentary rocks are often deposited in layers, and frequently contain fossils. Examples of sedimentary rocks include shale, sandstone, conglomerate, limestone, and chert. Sedimentary rocks are discussed in Chapter 9.
Metamorphic rocks are rocks that was once one form of rock but has changed to another under the influence of heat, pressure, or fluids without passing through a liquid phase (melting) (Figure 3-9). Examples of metamorphic rocks include slate, schist, gneiss, marble, quartzite, and serpentinite. Metamorphic rocks are discussed in Chapter 10.
These rock cycle diagrams illustrate how earth materials form and change over time. Both products (rocks and sediments) and processes (such as melting, cooling, erosion, and deposition) are illustrated. The passage of geologic time is an essential component, although some processes are much faster than others. Note that all these types of processes are taking place simultaneously, but at different locations on and within the planet. |
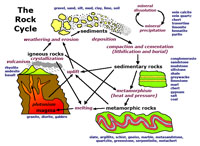
Fig. 3-3. The Rock Cycle: processes are in purple; products are in black and blue. |
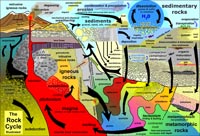
Fig. 3-4. Rock Cycle Illustrated. This version of the rock cycle shows more detail in graphic form. It is good to compare the two diagrams.
|
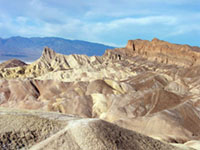
Fig. 3-5. The rock cycle includes examination of tectonics, forces that raise or lower the surface of the land and seafloor. Tectonic forces are responsible for creating mountain ranges and basins that fill with sediments. This view show the dynamic (changing) landscape of Death Valley, California. |
3.4
Preview of Selected Concepts Related To the Rock Cycle |
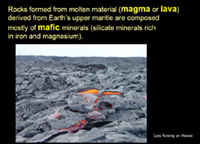 |
Fig. 3-10. Igneous rocks from the cooling of molten material (magma, lava) and are rich in silicate minerals. Molten material derived from deep in the Earth's mantle is typically enriched in iron- and magnesium-rich silicate minerals (and the rocks they form, these are called mafic or ultramafic). In contrast, magma and lava associated with continental crustal regions are enriched in felsic silicate minerals rich in silicon and aluminum (mostly feldspars) (The importance of felsic and mafic minerals are introduced below). This contrast between mafic and felsic materials is important to discussion about plate tectonics in Chapter 5. |
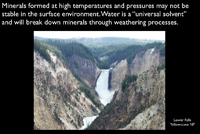 |
Fig. 3-11. Weathering of rocks and minerals: Water (H2O) occurs in rocks within the earth and is a primary chemical agent on the earth surface. Water is called the "universal solvent," dissolving and transporting material in solution, altering the chemical composition of mineral, and transporting sediment (erosion and deposition). Not all rocks and minerals behave similarly when subjected to weathering and erosion. Hard and durable minerals like quartz tend to resist weathering and erosion, and therefore can be carried long distances carried as sediments by flowing water or wind. |
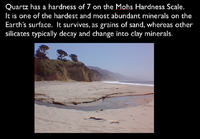 |
Fig. 3-12. Durability of quartz: Quartz an extremely abundant mineral in the Earth's crust. Quartz is also an extremely durable mineral (with a Mohs hardness of 7.0). As quartz-rich rocks weather and erode, minerals other than quartz tend to break down or decay into fine clay, whereas quartz grains tend to endure the abrasive processes while being transported by streams or battered by wave action on beaches. The finer clay materials get carried away by currents (water or wind). In contrast, the larger, more durable quartz grains become concentrated in the form of quartz-rich sand found on beaches and in desert sand dune fields. |
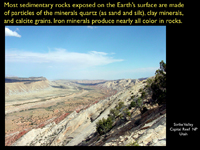 |
Fig. 3-13. Minerals in sedimentary rocks: Most sedimentary rocks are enriched in the minerals quartz, calcite, and some iron and clay minerals. Minerals with high hardness and low solubility are transported by erosion and deposited in sedimentary basins. Typically soft minerals that are highly soluble are dissolved and carried by surface and groundwater where most contributes to the saltiness of seawater. However, dissolved components in water can precipitate to form mineral cements, including like calcite (CaCO3), iron-rich minerals (hematite and limonite), and silica (quartz). The iron-mineral content in rocks are mostly responsible for the earth-tone colors they display. |
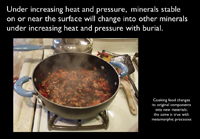 |
Fig. 3-14. Metamorphic processes cause changes in the mineral composition in rocks: Changes in heat, pressure, and exposure to fluids, over time, will change the mineral composition of earth materials, such as converting sediments into sedimentary rocks, and changing sedimentary and igneous rocks into metamorphic rocks. Conversely, exposing rocks to fluids—water and air at or near the surface—help to degrade rocks to form many kind into sediments. |
|
3.5
What is a rock formation?
Geologists who study and map the location of rocks in a region will assign them names and will described them by their composition and their geologic ages (if possible). A rock formation is a rock unit that is distinctive enough in appearance that a geologist can distinguish it from other surrounding rock layers. A named rock formation must also be thick enough and extensive enough to plot on a geologic map. Rock formations and geologic maps are a discussed in more detail below. Typically rock formations are named after a geographic feature or area associated with where a rock formation is exposed. for instance, the Kaibab Formation is named after the Kaibab Plateau where it crops out extensively. The name of the rock formation may also contain the name of it's dominant rock type, such as the Torrey Pines Sandstone Formation, a massive sandstone deposit of Eocene age that crops out in the sea cliffs at Torrey Pines State Beach Preserve in San Diego County, California.
Layered Versus Non-Layered Rocks
Large bodies of rocks typically display recognizable characteristics, most notable are characteristics of rock layers—called strata. Strata are layered rocks that are typically formed when an accumulation of rock material is deposited in a setting, then as time passes more layers accumulate on top. If cut and exposed by erosion or faulting, the layers appear as a series of beds. (A single bed is called a stratum). Rocks that display layering are called stratified; rocks that do not display obvious layering are called unstratified (Figures 3-15 to 3-18).
Some rocks are layered (or stratified), others are not. Sedimentary rocks and some volcanic deposits (lava flows and air-fall volcanic ash deposits) are examples of commonly stratified rocks. Examples of rocks that are unstratified are igneous rocks (like granite) that cooled and crystallized deep underground and did not develop layers. Also, rocks that have experienced extensive metamorphism and remelting may no longer preserve stratification. (As always, in geology, and science in general, there are sometimes exceptions to these rules!) |
3.6
Sedimentary rock formations display features that reveal information about the environments where they formed.
The term sedimentary facies is used to describe or interpret the origin of sedimentary deposits that reflect environmental conditions that existed at the time the sediments were deposited. Examples include: offshore mud facies, reef facies, beach sand facies, terrestrial swamp facies, etc. Facies reflect the character of a rock expressed by its formation, composition, and fossil content associated with the physical conditions at the time that they formed (Figure 3-13).
Sedimentary facies preserved in rock formations reveal much information about earth history, including how climates and geography have changed. They can reveal information about the timing of the formation of nearby mountain ranges, the movement of faults (associated with earthquakes), eruption of volcanoes, and the shifting of shorelines over time. |
Fig. 3-19. Sedimentary facies reveal information about past environmental conditions. |
3.7
Basic Geologic Principles
In 1669, Nicolas Steno first proposed several basic geologic principles that were later embellished by notable Scottish geologists, James Hutton and Charles Lyell. These basic principles are easily observed in geologic outcrops bearing layered rocks (strata), but have value for any number of scientific and technical applications beyond geology. Figure 3-20 illustrates basic geologic principles for Steno's three laws that are used in resolving the relative age of rocks and the order in which they formed or as geologic events occurred.
Steno's three laws are as follows:
Law of Original Horizontality—this law states that most sediments, when originally deposited, were laid down horizontally. However, many layered rocks are no longer horizontal—faulting and tectonic forces, and volcanic activity can deform rock layers
Law of Superposition—this law states that in any undisturbed sequence of rocks deposited in layers, the youngest layer is on top and the oldest on bottom, each layer being younger than the one beneath it and older than the one above it.
Law of Cross-Cutting Relationships—this law states that a body of igneous rock (an intrusion), a fault, or other geologic feature must be younger than any rock across which it cuts through.
Note that the boxes in Figure 3-20 illustrate vertical cross sections of what may exist below the surface. Cross sections are discussed more below. |
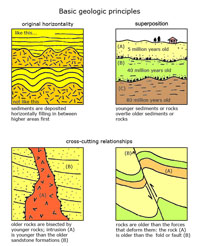
Fig. 3-20. Basic geologic principles illustrated. The four boxes represent vertical profiles below the ground surface, or a view such as may appear in a cliff on the side of a mountain or canyon. |
3.8
Unconformities: Gaps In the Geologic Record
Following on the Law of Original Horizontality and Law of Superposition, both Hutton and Lyell recognized erosional boundaries preserved between rock layers
representing gaps in the geologic record. They named these gaps unconformities (examples in Figure 3-21). An unconformity is a surface between successive strata that represents a missing interval in the geologic record of time. Unconformities show evidence that the Earth's surface may have been exposed to erosion for periods of time. Unconformities are produced either by an interruption in deposition or by the erosion of per-existing strata followed by renewed deposition. Note that unconformities can be complex. For example, may erosion may be taking place in one location in geologic time, where nearby or elsewhere sediments may have continued to be deposited and preserved. As a result, an unconformity in one location may span a different about of time in another location.
Several types of unconformity boundaries are recognized:
- nonconformity—an unconformity between sedimentary rocks and metamorphic or igneous rocks when the sedimentary rock lies above and was deposited on the pre-existing and eroded metamorphic or igneous rock
.
- angular unconformity—an unconformity where horizontally parallel strata of sedimentary rock are deposited on tilted and eroded layers, producing an angular discordance with the overlying horizontal layers
.
- disconformity—an unconformity between parallel layers of sedimentary rocks which represents a period of erosion or non-deposition.
- conformable boundary—an arrangement where layers of sedimentary strata are parallel, but there is little apparent erosion and the boundary between two rock layer surfaces resemble a simple bedding plane. There is typically little evidence to support a significant passage of time occurred at a conformable boundary.
|
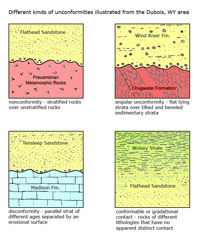
Fig. 3-21. Types of unconformities (boundaries between layered rocks representing "gaps" in the geologic record in a locality). The four boxes represent vertical profiles below the ground surface, or a view such as may appear in a cliff on the side of a mountain or canyon. |
Examples of unconformities and conformable boundaries in the Grand Canyon region of Arizona |
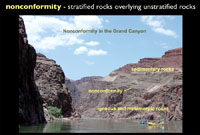 |
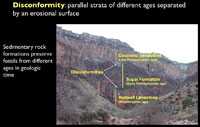 |
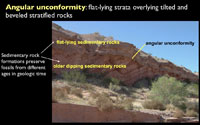 |
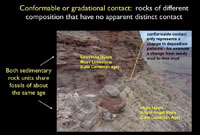 |
Fig. 3-22. Nonconformity in the Grand Canyon between Precambrian-age metamorphic rocks and overlying Cambrian-age sedimentary rocks. It is known as the "Great Unconformity" and is widespread across the region. |
Fig. 3-23. Disconformities between layers in the Grand Canyon. Disconformities occur between sedimentary rock formations of different ages and represent gaps of time spanning millions of years. |
Fig. 3-24. An angular unconformity occurs between sedimentary rocks of different ages. Uplift with folding and erosion of a rugged landscape occurred before the overlying sediments were deposited on the eroded suface. |
Fig. 3-25. Conformable or gradational contact between sedimentary layers. This type of boundary only reflect a change in how sediments were depositied—not necessarily representing a long gap in time. |
|
3.9
How Do Unconformities Form?
Unconformities are caused by relative changes in sea level over time. Wave erosion wears away materials exposed along coastlines, scouring surfaces smooth. On scales of thousands to millions of years, shorelines may move across entire regions. Erosion strips away materials exposed to waves and currents. New (younger) material can be deposited on the scoured surface. Shallow seas may flood in and then withdrawal repeatedly. Long-lasting transgressions can erode away entire mountain ranges with enough time.
A transgression occurs when a shoreline migrates landward as sea level (or lake level) rises.
A regression occurs when a shoreline migrates seaward as sea level (or lake level) falls (Figure 3-26).
Sea level changes may be caused by region uplift or global changes in sea level, such at the formation or melting of continental glaciers. Whatever the cause of sea level change, when sea level falls, sediments are eroded from exposed land. When sea level rises, sediments are typically deposited in quiet water settings, such as on shallow continental shelves or in low, swampy areas on coastal plains.
Some unconformities represent great gaps in time. For example, the Great Unconformity in the lower Grand Canyon illustrates where a great mountain range existed in the region during Precambrian before erosion completely stripped the landscape away back down, eventually allowing seas to flood over the region again in Cambrian time. The "gap in the geologic record" in some locations along the Great Unconformity represents billions of years (see Figure 3-21).
|
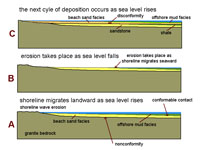
Figure 3-26. Unconformities can form by the rise and fall of sea level. Erosion strips away materials exposed to waves and currents. A rise in sea level causes a transgression which creates space underwater for sediments to be deposited. New (younger) material is deposited on the scoured surface. When sea level falls it causes a regression, and sediments are not deposited or are eroded away. |
3.10
How are the ages of rocks determined?
Geochronology is the branch of earth sciences concerned with determining the age of earth materials and events through geologic time.
How do geoscientists determine the age of rocks or fossils? How do they figure out how long ago and in what order did geologic processes or events take place? For instance how do they know how often a volcano erupts or how often earthquakes take place? Geologists now have many ways to determine the age of materials using relative dating and absolute dating methods.
Relative Dating
Relative dating is the science of determining the relative order of past events, without necessarily determining their absolute age (see below). Relative dating involved the study of fossils and the correlation or comparison of fossils of similar ages but from different regions where their age is known. Microfossils derived from sediments and cores from wells help in the subsurface exploration for oil and gas.
When studying an area where layered rocks are exposed, the Law of Original Horizontality dictates that the sedimentary layers were originally deposited as flat layers. The Law of Superposition dictates that if a series of rock layers are exposed, the oldest are on the bottom of the stack. That is true, unless the sequence of rock layers have been disturbed by some later geologic even. It that case, the Law of Cross-Cutting Relationships dictates that the rocks will be older than the forces that later changed them. Examples of forces that change landscapes include movement of faults, or the tectonic folding of rock layers, or an intrusion of igneous material, such as the formation of a volcano.
Example! Use these rules to interpret this general vertical cross section of a landscape (Figure 3-27). The diagram could represent a road cut along a highway or a wall on the side of a canyon. The Law of Original Horizontality and Law of Superposition suggest that layer C (layers of shale) was deposited before layer B (beds of limestone), which was deposited before layer A (beds of sandstone). The Law of Cross-Cutting Relationships dictates that next thing to happen was that feature D (an igneous intrusion) cut across the sedimentary layers. After that a fault (feature E) broke through all the older materials. The final thing to happen was erosion of the landscape down to partially expose some of the features on the surface. Note also that the boundaries between layers C and B, and A and B may represent unconformities (possible gaps in time).
Relative dating only can be used to sort the exposed visible features in the order that they formed (example in Figure 3-28).
Fossils and Relative Dating
Sedimentary rock layers of different ages often look very similar. Conversely, sedimentary rock layers of similar ages may appear very different in other locations. Sometimes fossils preserved in sedimentary layers are very useful for correlating rock layers from one area or region to another. Paleontologists have extensively studied fossil found in sedimentary rock formation of all geologic ages around the world. Certain "index fossils" are both abundant and widely distributed through sedimentary rocks of relatively limited geologic time ranges.
Unfortunately, many rocks formation do not contain fossils, or the fossils they may contain are very rare or poorly preserved. Metamorphic and igneous rocks do not contain fossils. In many cases, the exact age of each of the rock units is unknown until it can be confirmed by other means involving absolute dating methods (discussed below). |
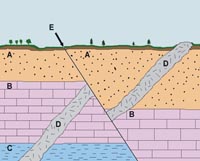
Fig. 3-27. Applying basic geologic principles: Laws of Original Horizontality, Superposition, and Cross-Cutting Relationships explain the order of this diagram with the order of formation: rock units C, B, A, D, then E (followed by surface erosion).
Fig. 3-28. Rock layers like these in Utah record information about 100 million years of Earth history exposed in the region. These rocks were originally deposited horizontally but then were later folded upward: oldest rocks on the right; youngest on exposed on the mesa top on the left. |
3.11
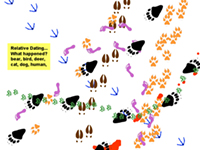
Fig. 3-29. Relative Dating Exercise #1. Tracks left in the mud along a river bank include a bear, bird, deer, dog, bobcat, and human (and blood). What happened, and in what order? |
Try Relative Dating (sort out the visually available clues in a map view).
The basic geologic principles have many applications to interpreting the order of events on many scales, ranging from very large (like the Grand Canyon or the geologic history of parts of the Rocky Mountains), to small scale, like interpreting the order of footprints along a lake shore (as illustrated in this exercise).
Geologists use basic geologic principles to study geology map (as illustrated below), and use them to unravel the complex history of an area, such as planning tunnels in large underground mining operations, or for exploring for oil and gas, and deep-water resources.
Try interpreting the order of events in the map-view diagrams involving animal trackways (Figures 3-29 and 3-30). Notice that some tracks overly (are superimposed) on other tracks. Sometimes things aren't as clear as they seem, but inferences can be made.
Basic geologic principles are used to interpret the geologic history of an area. See examples in Figures 3-31 to 3-34.
|
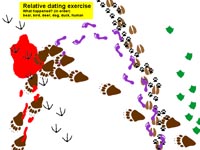
Fig. 3-30. Relative Dating Exercise #2. Tracks left in the mud along a river bank include a bear, bird, deer, bobcat, duck, and human. Can you figure out the chronology of events in this nature scene? |
3.12
Examples of how the basic geologic principles can be applied to relative dating on rock outcrops. |
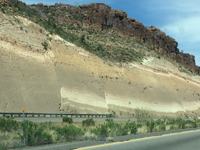 |
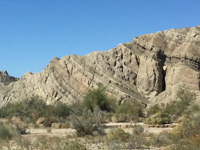 |
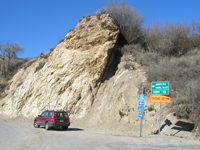 |
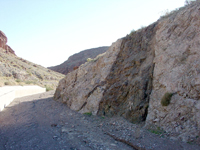 |
Fig. 3-31. Minor faults cut though layers of volcanic ash beds and sedimentary rocks, exposed along I-40 near Kingman, AZ. |
Fig. 3-32. Folded layers of sedimentary rocks exposed near the San Andreas Fault in Box Canyon near Mecca, CA. |
Fig. 3-33. A fault cuts trough sedimentary rocks along Arroyo Seco Canyon in the Santa Lucia Range in Monterey County, CA. |
Fig. 3-34. A basalt igneous intrusion cuts through older volcanic rocks in Lake Mead National Recreation Area, NV. |
|
In Figure 3-31, the Laws of Original Horizontality and Superposition show a series of sedimentary layers and volcanic ash bed deposited from oldest (on the bottom) to a lava flow (on the top of the cliff). The Law of Cross-Cutting Relationships shows that the layers were offset by faulting after they were deposited as layers.
In Figure 3-32. the Laws of Original Horizontality and Superposition indicate that this series of sedimentary layers were deposited horizontally before tectonic forces pushed up these layers located near to the San Andreas Fault. Therefore, the San Andreas Fault must be younger than the sedimentary layers.
In Figure 3-33, the Law of Cross-Cutting Relationships suggests that the fault in the picture is younger than the sedimentary rock formation on either side of the fault. However, the age of the two rock formation on either side would have to be determined by some other means (such as by the fossils they may contain).
In Figure 3-34, the Law of Cross-Cutting Relationships suggest that the dark, basalt igneous dike is younger than the pink volcanic rocks on either side, however, the exact age of the volcanic rocks would have to be determined by other means. |
3.13
Cross Sections
Cross sections are important tools for relative dating!
A geological cross-section is a graphic representation of the intersection of the geological features in the subsurface with a vertical plane. Where the vertical plane intersects the surface is typically shown as a line on a map. Like geologic maps, cross sections show different types of rocks, their structure, and the geometric relationship between them are represented. Note that geologic cross sections are made by using available mappable features found on the surface or interpreted from data about the subsurface. Natural cross sectional views are sometimes possible along canyon high walls or along steep mountain range front (Figure 3-35). How most subsurface data derive or imaged through geophysical methods, such as by seismic data (by earthquakes or man-made explosions), by measurements of gravity, magnetism, electrical resistivity, or information derived from wells such as core sample, radiation measurements, or other geophysical methods.
|
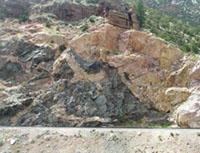
Fig. 3-35. A wall of rocks exposed in a cliff illustrate the vertical view illustrated by a cross section. This cliff shows igneous intrusions exposed in Wind River Canyon, Wyoming. |
3.14
Geophysical Methods Used To Make Cross Sections
Geologists have a variety of geophysical methods to collecting information about geologic features underneath the surface. For example, geologists use use sound (shock waves produced by explosives or machines making sounds to penetrate the ground). Other geophysical methods collect measurements using electricity and radar to penetrate the ground,
and measurements of variations in Earth's gravity and magnetism. In addition, geologists use data from wells drilled in an area to add information to cross sections.
Seismic Reflection Techniques Produce Cross Sections Used For Subsurface Exploration
Seabed exploration produces cross-sectional seismic profiles, raw data that are converted to cross-section diagrams (Figure 3-36). Modern systems produce views that are in three dimensions.
Geologists study cross sections created by geophysical exploration methods. Figure 3-37 is an example of a seismic profile showing the location of exploratory wells.
|
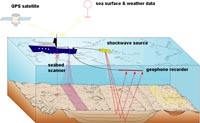
Fig. 3-36. A marine seismic reflection profile expedition.
Fig. 3-37. A seismic reflection profile data is a cross section. |
3.15
Relative Dating Using Cross Sections - Examples |
Geologists search for locations on the landscape to try to resolve the geologic history in a region. In most places, this is not an easy task because the landscape feature often cover or disguise geologic features. In most places, soil and vegetation cover the bedrock, and private property designations often prevent geologists from gaining access to suitable outcrops where fresh rock material is exposed. Geologist have to work with regional government and land management companies in order to gain access to land and construction sites, and this is often quite time consuming. However, in many regions, only a few good outcrops, road cuts, or stone quarries provide access to enough detail for field geologists to reconstruct the geologic history of a region, and make assessments of potential geologic hazards, such a active fault zones (and earthquake history), landslide hazard-prone areas, and the history of volcanic eruptions in a region. Ideal locations for study geology include exposures in canyon walls or fresh road cuts, or sea cliffs where wave erosion create fresh exposures of bedrock geology. Figure 3-38 illustrates a sea cliff in Encinitas, California where an angular unconformity is locally exposed between massive layers of sedimentary rock. The sea cliff exposure provides a natural cross section view of rocks hidden in the subsurface. Sites like this can be used to interpret the geologic history of this part of the coastline. |
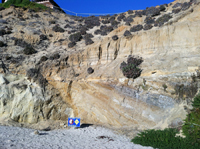
Fig. 3-38. Sedimentary rocks and an unconformity exposed in a sea cliff at Fletcher Cove in Encinitas. California |
3.16
Figure 3-39 illustrates a cross section of a hypothetical landscape with an active volcano. Beneath the surface in this landscape are a mix of layered and non-layered rocks. Letters correspond to different features including a fault, unconformities, and the active magma chamber feeding lava to the volcano erupting on the surface. Examine the cross section to see the chronology of the formation of geologic features, from oldest to youngest represented by letters A to Q. [Be sure to review Figures 3-20 and 3-21, and open the larger view of Figure 3-39 while you review the information below.]
- Letter A represents ancient non-stratified rocks (possibly a mix of metamorphic and igneous rocks). These ancient rocks may have been an ancient mountain range that later wore down by erosion over time.
- Letter B represent a unconformity (an erosional gap in time) between the ancient rocks below and layer C, a layered sedimentary bed on of the older rocks (A). (The letter B is technically a nonconformity between stratified and non-stratified rocks).
- Sedimentary layers D and E were deposited (originally horizontal beds) on top of layer C.
- Letter F point to the unconformity that is actually younger than the unconformity at letter B! This unconformity represents a period of uplift and erosion before sedimentary layer G was deposited. This unconformity is a nonconformity or an angular unconformity depending where you examine it.
- Sedimentary layers G, H, I, J, and K were deposited in that order, following the Laws of Superposition and Original Horizontality.
- Another period of uplift occurred. During this time, a fault formed (Letter L). In addition layers G, H, I, J, and K were gently folded (warped) upward by renewed tectonic forces.
- Letter M represents another unconformity. This unconformity beneath layer N tells us that the fault (L) formed before sedimentary layer N was deposited on the eroded surface and younger than layer K. Note! Without more information we can only know that the fault (L) is younger than layer I but is younger than layer N.
- Sedimentary layer O horizontally rests on top of layer N.
- Finally, igneous activity produced volcano (P). Molten material is still flowing from the magma chamber (Q) to produce the active eruption on the volcano.
|
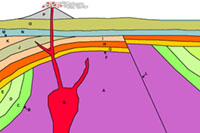
Fig. 3-39. A hypothetical cross section showing layers beneath a landscape with an active volcano in a region where mountain building has occurred in the ancient past.
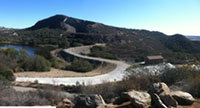
Fig. 3-40. The region around the extinct Calavera Hills Volcano in Carlsbad, California (San Diego County) has a similar geologic history as illustrated in Figure 3-39.
|
3.17
When working with cross sections is is often easiest to start from the "top" (youngest rocks) and work backwards in time to the "bottom" (oldest rocks). Then, work things out in reverse older to tell the geologic history of a region. Be sure to examine the geologic time scale to understand the ages of the rocks in this cross section.
Example of a Cross Section of the northwestern end of the Wind River Basin near Dubois Wyoming.
Figure 3-41 is a satellite image map of northwestern Wyoming. Grand Tetons National Park is in the northwest corner, the Wind River Basin and Wind River Mountains are in the southeast corner.
Figure 3-42 is a view of a mountainside on the eastern flank of the Wind River Mountains in Torrey Canyon (near Dubois, a small town in northwest Wyoming—a location where many geology students go to learn about geology and field mapping of geologic features in the region; Torrey Canyon is located along red line just south of Dubois on Figure 3-41).
Figure 3-43 is a generalized cross section through the Wind River Mountains, Wind River Basin, and Absaroka Mountains (including Dubois, Wyoming). Note the location of Torrey Canyon on the cross section. Also note that the view of the cross section is looking toward the southeast, perpendicular to the red line on the map in Figure 3-40. Here is how the regional geologic history can be interpreted from this cross section. Key word clues are highlighted.
1) The oldest rocks are the highly deformed very ancient Precambrian metamorphic rocks (dark red).
2) The metamorphic rocks are cross cut by Precambrian-age intrusive igneous rocks (light purple).
3) Younger intrusive igneous rocks intruded the older intrusive igneous rocks (pink).
4) The landscape was eroded down before sediments were deposited on top (creating a major unconformity).
5)
Sedimentary rocks of Paleozoic age formed from sediments deposited when seas intermittently covered the region, these sediments were originally deposited horizontally (light green).
6) Sedimentary rocks of Mesozoic age were deposited were deposited on top (superimposed) of the Paleozoic-age sedimentary rocks (darker green).
7) Faults (shown with lines and arrows showing relative movement of rocks) cross cut through Precambrian, Paleozoic, and Mesozoic age rocks. Note that the faults do not cut through the younger Cenozoic-age valley-fill sediments (thin yellow layer with spots). Other faults in the region probably formed at this time (this happened during the early uplift and formation of the Rocky Mountains during the Laramide Orogeny).
8) Volcanic rocks were deposited on top of the eroded landscape of the older mountains (light brown with V symbols).
9) A younger volcanic intrusion cross cuts through the older rocks (light red).
10) Cenozoic-age sedimentary cover fills the Wind River Basin (red spots in the yellow valley fill deposits show that some of the sediment came from #9 [eroded volcanic rocks]).
11) Uplift and erosion in the region over that last few million years exposed many of the geologic features now exposed throughout the landscape in the Wind River Valley and surrounding landscape.
|
Fig. 3-41. Satellite view of northwestern Wyoming showing location of cross-section line in Fig. 3-43.
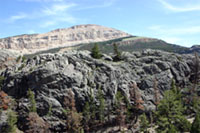
Fig.
3-42. View of Torrey Canyon in the Wind River Mountains near Dubois, Wyoming.
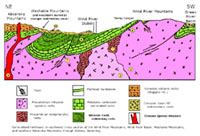
Fig. 3-43. Cross section of the Wind River Mountains and Basin region, Wyoming. |
3.18
Cross Section of the Little Rocky Mountains Region of Montana
Figure 3-44 illustrates a cross section trending north-to-south across a mountain range in central Montana. The diagram shows two large (red) igneous intrusions that formed when magma pushed up and created two large bubble-like bodies (magma chambers) beneath older layers of sedimentary rocks (blue, orange, and brown). Erosion long after the igneous activity stripped away rocks, exposing steeply dipping layers of layered sedimentary rocks exposed on the flanks of the high peak of the mountain range.
|
Fig. 3-44. Cross section of Little Rocky Mountains, Montana. |
3.19
Cross Section of the Southern San Francisco Bay Region
Figure 3-45 shows a cross section through the Santa Cruz Mountains, Santa Clara Valley, and Diablo Range in the vicinity of San Jose California. The cross section represents a distance of about 30 miles, the vertical is highly exaggerated to highlight the landscape features.
The cross section shows a complex series of faults that separate great blocks of rocks of mixed ages and compositions in the region. In this case, relative dating at least shows that the faults are younger than the rocks they cut through. However, other means would be necessary to explicitly date the age of the rocks and the (younger) faults. |
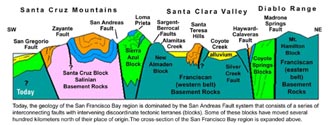
Fig. 3-45. Cross section of the South Bay region, California showing major faults between great blocks of rocks of different age and origin.
|
3.20
Cross Section - Relative Dating Interpretation Exercise |
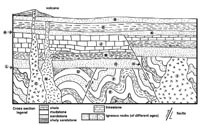
Fig. 3-46. Geologic cross section exercise. |
Use the Laws of Original Horizontality, Superposition, and Cross-Cutting Relationships and unconformities to interpret the order of the formation of features illustrated in this hypothetical cross section (Figure 3-46). Be sure to review Figures 3-20 and 3-21,
Note that a legend on the bottom of the cross section indicates different kinds of rocks illustrated in the cross section.
Letters on the cross section indicate geologic features including sedimentary rock layers, igneous intrusions, faults, and unconformities (lines representing periods of erosion and non-deposition).
Create a list (letters A to P) of the "events" in order as they occurred, from oldest to youngest.
The answers to this exercise are posted at the end of this chapter (below). |
3.21
Absolute Dating Methods
Absolute dating is a general term applied to a range of techniques that provide estimates of the age of objects, materials, or sites in real calendar years either directly or through a process of calibration with material of known age.
There are many methods of absolute dating rocks or other ancient materials. The methods of absolute dating used depends on whether suitable sample are available for testing.
One variety of absolute dating methods involves the study of the decay of radioactive isotopes. The original isotope is the "parent" which through radioactive decay becomes the "daughter" isotope. Commonly referenced studies of absolute dating utilize the radioactive decay of 238U (unstable uranium isotope) into 206PB (stable lead isotope); or 40K (unstable potassium isotope) into 40Ar (stable argon isotope). Note that here are many other absolute dating methods. Perhaps most important is radiocarbon dating which utilizes the decay of 14C (unstable carbon isotope) into 14N (stable nitrogen isotope). Dating of materials that contain naturally-occurring radionuclides (radioactive isotopes) is possible because the rate of decay of the radionuclides are known. The radiation decay "clock" starts the moment a mineral in a rock forms (or for 14C when an organism dies).
Figure 3-47 shows information about several absolute dating methods. Each method uses different parent/daughter isotopes. Each method has a suitable age dating range and materials that can be dated (such as minerals, shells, organic mater, etc.). It is important to note that today there are dozens of other absolute dating methods using a variety of technological innovations. A half-life is when only half of a parent radionuclide remains. The next half-life is when only a quarter of the original parent radionuclide remains, and so on. Age determinations can be determined by comparing the percentage of the radionuclides in a new "fresh" sample with the percentage in the old sample material being tested.
|
Fig. 3-47. Absolute dating methods. Different isotopes are used to study different materials and geologic time ranges. This diagram illustrates the concept of half lives of decaying radioactive elements commonly used in many absolute dating methods. |
3.22
Absolute Dating of Moon Rocks
Six Apollo Mission space craft landed to the Moon (1969-1972). The missions returned with about 820 pounds (382 kilograms) of moon rocks. Figure 3-48 shows one of the largest samples, a medium-grained olivine basalt collected by Apollo 15 astronaut, Dave Scott, who collected the sample from the rim of Hadley Rille on August 2, 1971. The collection of the moon rocks was a driving force for finding new absolute dating methods for rocks, like these very-expensive samples. Absolute dating studies of the moon rocks show that most of the sample range in ages between about 3.16 billion years for rocks from the Moon's Mar regions, to as old as 4.4 billion years for older rocks exposed in the more ancient Lunar Highlands regions. The older moon rock samples are older than any rocks yet found on Earth.
Absolute dating studies of the sample shown in Figure 3-48 has resolved that the rock came from an eruption of molten material from the ancient Moon's mantle about 3.3 billion years ago. The current age of the origin of the Moon is 4.51 billion years. |
Figure 3-48. A sample of a moon rock collected on the Apollo 15 Mission of 1971. |
3.23
Radiocarbon Dating
14C (isotope carbon -14) is a unstable radioactive isotope of the element carbon (a radionuclide). Radiocarbon dating involves using ratios of the isotopes of radioactive isotope14C to stable isotopes 12C and 13C derived from buried or isolated organic or carbonate materials. Figure 3-49 illustrates the science behind radiocarbon dating. The half life of 14C [unstable isotope carbon-14] is about 5,730 years. Radiocarbon dating has extensively used in archaeological investigation and the study of climate change over the last several hundred thousand years, and precision methods now available make radiocarbon dating highly reliable. Radiocarbon dating is highly effective for extracting ages of organic materials (bone, tissues, wood, etc.) that have been isolated from the atmospheric interactions by burial. Radiocarbon dating is widely used and has proven particularly effective for dating materials from ancient archaeological sites to date human activities going back for many thousands of years.
|
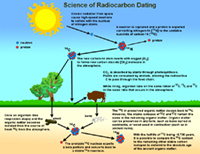
Fig. 3-49. The science behind the radiocarbon absolute dating method. |
3.24
Answer to the Cross Section Exercise in Figure 3-46.
The
order of formation (from oldest to youngest) is: P - E - F - D - I - L - O - M - J - H - A - K- G - N - B - C
Note that it is sometime easiest to start at the top and work backward on such diagram before you recreate the "geologic history" of an cross section. Here is what happened here (starting from oldest to youngest).
Sedimentary layers were deposited (following the Laws of Superposition and Original Horizontality).
1. P — shaly sandstone was deposited as a horizontal layer.
2. E — mudstone was deposited as a horizontal layer.
3. F — layers of sandstone were deposited as horizontal layers.
4. D — more mudstone and shaly sandstone layers were deposited as horizontal layers.
5. I — represents a fault that formed during a period of mountain building where the older layers P, E, F, and D were folded, faulted and intruded by igneous rock.
----- The law of cross-cutting relationships depicts that the fault [ I ] is younger than sedimentary layers P ,E, F, and D.
6. L — represents an unconformity, mountain building had ended, the landscape had eroded flat over a long period of time.
7. O — sandstone was deposited as a horizontal layer on top of the ancient eroded landscape surface.
8. M — shale was deposited as a horizontal layer on top of the sandstone [M].
9. J — layers of limestone were deposited as horizontal layers on top of the shale [J].
10. H — a fault formed, cutting through all the previously formed rock layers - as defined by the law of cross-cutting relationships.
11. A — the letter A represents an unconformity - layers of limestone on the right side are thinner, suggesting there was either erosion and/or non-deposition.
12. K — a layer of sandstone was deposited above the unconformable surface.
13. G — a layer of shale was deposited on top of the sandstone.
14. N — an igneous intrusion cut through all previously formed rock units, possibly forming a volcano.
15. B — after the igneous intrusion {N] formed, erosion stripped away any evidence of a possible volcano, and a layer of sandstone [B] was deposited.
16. C — another igneous intrusion formed, resulting in eruptions that formed the volcano still present on the land's surface.
Other things to consider on this cross section exercise.
Note the igneous rocks with X markings on the right side of the diagram are truncated by the unconformity [L] - the same as the fault [ I ]. Which one is older? It is impossible to tell — they are "relatively" the same age. In addition, all the boundaries between the rock formations could be unconformities, but their significance in age differences would have to be determined by some other means.
Unconformity [L] clearly demonstrates that it is an unconformity represents a long period of time passage. In some places it is an angular unconformity; over the intrusion [ with the X markings] it is a nonconformity.
The unconformity marked as letter A is somewhat problematic. It truncates the fault (letter H). The problem is that it would take further examination to determine whether or not fault H was forming at the same time that limestone J was being deposited!
The boundary between sedimentary layers G and B is also an unconformity that represents a significant passage of time — at least long enough for a volcano to form and then be stripped away by erosion.
|
|